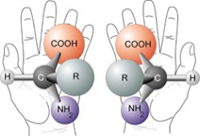
Advances in the field of amino acid geochemistry were recently reviewed (Goodfriend et al., 2000), twenty years after the publication of the original compilation on the topic (Hare et al., 1980). Among the most well-studied reactions involved in amino acid diagenesis in fossils is amino acid racemization (
AAR). The principles of
AAR for geological purposes have been summarized by Miller and Brigham-Grette (1989), Wehmiller (1993), Rutter and Blackwell (1995), and Wehmiller and Miller (2000), among others. Fossil remains of biogenic minerals contain trace quantities of indigenous organic matter, which is preserved for long periods of geologic time. After synthesis by an organism, proteins and their constituent amino acids degrade through a complex series of biogeochemical reactions (Mitterer, 1993). The extent to which these reactions have progressed in a fossil is proportional to the length of time elapsed since the organism lived and to the ambient temperature of the reaction medium. The most reliable of the complex network of diagenetic reactions is amino acid racemization, which involves the interconversion of L-amino acids to their D-isomeric configurations. Most living organisms use amino acids exclusively of the L-configuration. Upon death and removal of biologic constraints, the L-amino acids begin to racemize to their D-configuration. The abundance of D- relative to L forms (D/L) defines the extent of racemization for a particular amino acid. The ratio increases with time and temperature until the rate of formation of D-amino acids is compensated by the reverse reaction, and the ratio reaches an equilibrium value of 1.0.
Two general approaches are used to convert D/L ratios to an absolute time scale: The first is a calibrated approach in which the D/L ratios are used to interpolate between, or extrapolate beyond, the known ages of independently dated samples within a restricted geographic/ oceanographic area, where temperature histories are similar. In the second approach, the effects of time and temperature on the extent of racemization are determined in modern shells subjected to high-temperature laboratory experiments, and in Holocene samples whose ages are known from 14C analysis and whose temperature history can be inferred based on instrumental data. This relation, together with a model of racemization kinetics, is used to calculate the age of a sample if its D/L ratio and temperature history are known. In practice, some combination of the two approaches is typically employed. For example, a kinetic model is used to estimate the age of an undated sample by extrapolating beyond a calibration point, or a calibration curve developed for one thermal regime is applied to another using a kinetic model to adjust the reaction rate for differences in site temperature.
Because the extent of racemization is dependent on both temperature and time, the average postdepositional temperature ("effective diagenetic temperature"; Wehmiller, 1977) can be determined from the D/L ratio if the sample age is known independently. By integrating the entire postdepositional temperature history of a deposit, amino acids record long-term climate changes, as compared to the geologically instantaneous paleoenvironmental evidence contained within the deposits. Unlike many other proxy indicators of paleoclimate, amino acid paleothermometry is based on processes that are independent of precipitation changes, and therefore allow paleotemperatures to be calculated uniquely. The principles and procedures used to derive paleotemperature from amino acid data are well documented in the literature (e.g., McCoy, 1987; Oches et al., 1996; Miller et al., 1997; Kaufman, 2003b).
References
Goodfriend, G.A., Collins, M.J., Fogel, M.L., Macko, S.A., and Wehmiller, J.F., eds., 2000, Perspectives in Amino Acid and Protein Geochemistry: New York, Oxford University Press, 366 p.
Hare, P.E., Hoering, T.C., and King, K., Jr., eds., 1980, Biogeochemistry of Amino Acids: New York, Wiley, 558 p.
Kaufman, D.S., 2003b, Amino acid paleothermometry of Quaternary ostracodes from the Bonneville Basin, Utah: Quaternary Science Reviews 22, 899-914.
McCoy, W.D., 1987, The precision of amino acid geochronology and paleothermometry: Quaternary Science Reviews 6, 43-54.
Miller, G.H., and Brigham-Grette, J., 1989, Amino acid geochronology: resolution and precision in carbonate fossils: Quaternary International 1, 111-128.
Miller, G.H., Magee, J.W., and Jull, A.J.T., 1997, Low-latitude glacial cooling in the Southern Hemisphere from amino acids in emu eggshells: Nature 385, 241-244.
Mitterer, R.M., 1993, The diagenesis of proteins and amino acids in fossil shells, in Engel, M. H., and Macko, S.A., eds., Organic Geochemistry. New York, Plenum Press, 739-753.
Oches, E.A., McCoy, W.D., and Clark, P.U., 1996, Amino acid estimates of latitudinal temperature gradients and geochronology of loess deposition during the last glacial maximum, Mississippi Valley, United States: Geological Society of America Bulletin 108, 892-903.
Rutter, N.W., and Blackwell, B., 1995, Amino acid racemization dating, in Rutter, N.W., and Catto, N.R., Dating Methods for Quaternary Deposits, Geological Association of Canada, Geotext 2, 125-167
Wehmiller, J.F., 1977. Amino acid studies of the Del Mar, California, midden site—Apparent rate constants, ground temperature models, and chronological implications. Earth and Planetary Science Letters 37, 184-196.
Wehmiller, J.F., 1993, Applications of organic geochemistry for Quaternary research; Aminostratigraphy and aminochronology, in Engel, M.H., and Macko, S.A., eds., Organic Geochemistry: New York, Plenum Press, 755-783.
Wehmiller, J.F., and Miller, G.H., 2000. Aminostratigraphic dating methods in Quaternary geology, in Noller, J.S., Sowers, J.M., and Lettis, W.R., eds., Quaternary geochronology: methods and applications, American Geophysical Union, Washington D.C., 187-222.